1 Foundational Technology Layers
1.2
Components, Modules and Systems Integration
Development and production of smart electronic components and systems (ECS) requires physical and functional integration (PFI) of several functionalities into a new physical entity at component, module and system levels. Therefore, PFI is one of the essential capabilities required to maintain and improve the competitiveness of European industry in the application domains of smart systems. Although in practice PFI is often application specific, the materials, technologies, manufacturing and development processes that form these domains are generic and should be standardized, interoperable and reconfigurable where possible. Particularly, heterogeneous integration of devices and components fabricated with separate and different fabrication processes is key to PFI. This Chapter deals with approaches beyond the semiconductor technologies, material families and compact system on a chip (SoC) integration which are elaborated in the Process Technology, Equipment, Materials and Manufacturing (PTEMM) Chapter.
In the development of ever better smart systems and innovative products, heterogeneous integration becomes more and more important at every level of integration, from semiconductor SoC to System-in-Package (SiP) and ultimately to larger modules and systems. The importance of SiP technologies in integration terms is emphasized by the treatment of SiP in both this and the previous chapter from their specific point-of-view. Particularly, in this Chapter alternative technologies (such as additive manufacturing), complementary materials (both at the functional and structural/substrate level) and heterogeneous approaches to assembly, integration and advanced packaging are considered. The term heterogeneous integration is used in its widest meaning: components should be taken to mean any unit, whether individual die, MEMS device, passive component or assembled package, that are integrated into higher order single components, modules or systems. Developments of heterogeneous integration technologies and platforms include also flexible electronics and photonics solutions.
In addition to the usual silicon-based semiconductor technologies, smart components, modules and systems require the following characteristics:
- A combination of device architectures: sensors (including all kinds of image sensors), actuators, energy generators, storage devices, MEMS/NEMS, MOEMS, LAE, processors and communication interfaces.
- Heterogeneous integration technologies at the component, module and system level, utilizing multi-physics/multi-domain approaches, e.g. nano-electronics, micro-electro-mechanic, thermoelectric, magnetic, photonic, quantum effect, micro-fluidic, acoustic, radiation, RF, and bio- and chemical principles. A multitude of processes: micro and nanotechnologies, additive manufacturing, printing, lamination, assembly and interconnection technologies, as well as hybrid combinations.
The methods, processes and schemes required for the design, production, assembly and testing of the various components, modules and systems and their integration need to be devised with appropriate quality, reliability as well as scalability (appropriate unit cost, also including life cycle considerations) and sustainability (circular economy, CO2 footprint, efficient use of resources).
Smart components, modules and systems are the key enabling link between basic technologies, e.g. semiconductor or interconnection technology, and key applications as described in the Application Chapters. They open the way for widespread use in all application domains by integrating functionalities such as intelligence, sensing, communication and control, even in the smallest devices, through simultaneous development and co-design with Embedded Software and System of Systems (SoS) technologies and with support from cross-sectional technologies: Artificial Intelligence (AI), Connectivity, Architecture and Design, and Quality, Reliability, Safety and Cybersecurity.
Considering the new requirements imposed by modern and future smart systems, mastering the integration technologies at component, module and systems levels, is a significant capability of European industries. Such a strength needs to be sustained and reinforced to ensure Europe’s leading position in smart systems engineering, as well as to bring innovations into real-life reliable and sustainable products, services and markets.
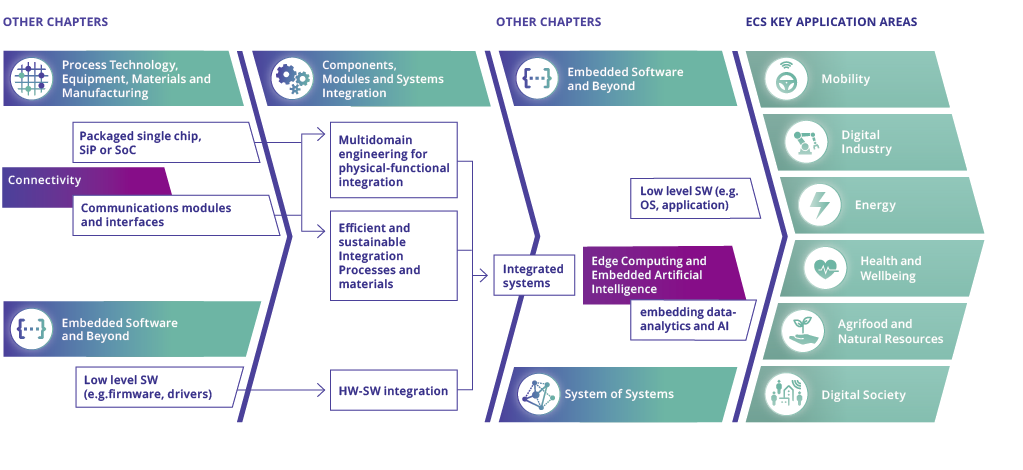
Societal benefits of smart components, modules and systems stem from the applications that they enable, as described in the Application Chapters. Improved integration technologies and miniaturization, together with cost-, energy- and resource-efficient and eco-friendly manufacturing, will make future applications affordable for the broader public, and support sustainability of products and production technologies, enabling responsible use of resources, e.g. by means of assisting in the development of a circular economy, in alignment of the European Green Deal31 and UN sustainable development goals32.
Figure F.11 defines an integrated smart system showing its components and modules, while interacting with the natural, man-made and any sort of environment. Smart systems integrate sensing and/or actuation as well as signal processing to enable actions. Smart systems utilize multifunctional perception, and are predictive, configurable, contextual and adaptive.
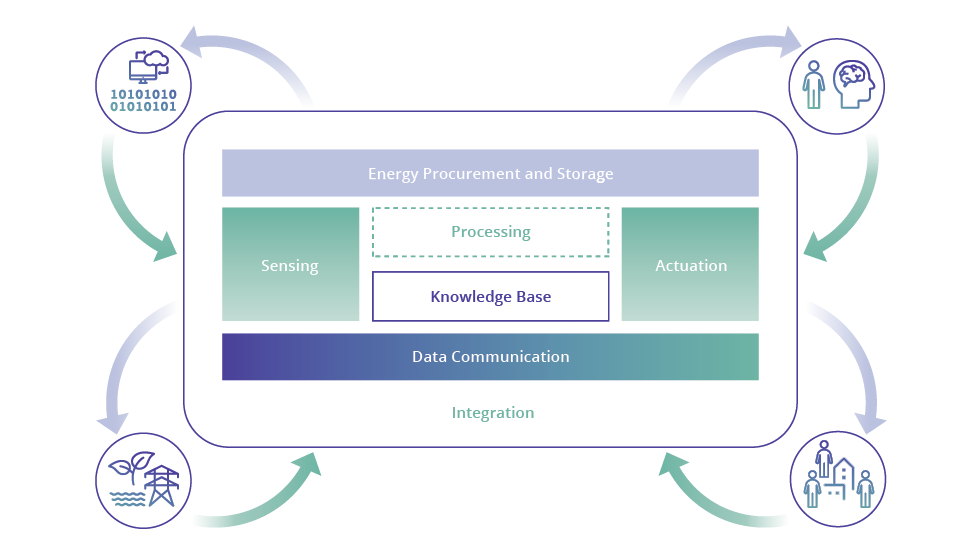
The Covid-19 pandemic has demonstrated the critical role that smart components, modules and systems can play for the world’s security and health. Key topics here range from an acceleration in the analysis of DNA samples, the availability of automated medical support and diagnosis tools, as well as tracking systems for tracing and controlling the spread of the disease, not to mention the moral and physical assistance that smart devices have provided to quarantined people through cellular networks and the internet.
The Internet of Things (IoT) is one of the main technologies enabled by smart components, modules and systems. It supports such game changers as virtual reality (VR), augmented reality (AR), extended reality (XR), digital helpers, and spread use of AI and edge computing. These elements are pivotal for optimized data collection and profitable machine-to-machine (M2M), human–machine interface (HMI) and human–computer interaction (HCI), bringing smartness to human activities (smart cities, smart transportation, smart grids, smart manufacturing, etc.) and human health and wellbeing (e-health, m-health, implants, ingestibles, wearables, personalized medicine, inclusion of people with a disability, etc.).
Another major application is digital industry. Smart systems harness data, extract information, distill knowledge and convert it into actions and/or provision of improved decision support. This is achieved by integrating components and modules for data acquisition and context-based actions, signal conditioning and data analysis, and by communicating elements to organize collaborative, adaptive and self-repairing networks. In offering alternative access via the cloud to data processing and knowledge extraction engines, smart components, modules and systems enable the deployment of edge computing, thus reducing the demands on communication bandwidth and system-level power consumption. However, this also creates challenges for the sustained self-powering of edge devices of increasing complexity, and the need for such power demands to be carefully managed and limited, using ambient energies where possible. Software (SW) integrated into such devices needs to be considered very carefully. How, where and when data is gathered, processed and transmitted has major ramifications on the peak and average power consumption not only of the edge devices but also the gateways, servers and the cloud. The most energy efficient manner possible should be found to maximize battery life and reliability. Having billions-connected IoT devices will increase the load on our IoT infrastructure at all levels so the overall architecture in inter-play between the entire system components needs to carefully considered in terms of power, security, latency, processing capability, etc. Resolving this challenge requires not just technology advances, but also close coordination and collaboration between all the “power IoT” stakeholders based on realistic targets and expectations.
Technology advances enabled by components, modules and systems integration are as follows:
- Greater performance and digital configurability of sensors, analogue and RF components.
- Technology integration of electronics, photonics, chemical/biochemical interaction, fluidics for multifunctional smart systems.
- Embedded intelligence in the ECS, typically realized by a combination of hardware and software components, leading to higher functionality, improved interoperability, less demanding or more natural interfacing, both for machine-to-machine interfacing, human-to-machine interfacing (e.g. haptic interfaces) and decentralized signal and information processing, i.e. edge AI.
- Greater performance in power electronics components through an impact on the thermal characteristics and higher power density and electromagnetic interference (EMI) performance of components, modules and systems.
- Reducing energy consumption of electronic components, without decreasing component performance (e.g. in the detection limit of MEMS/NEMS sensors), on all levels and functions: component, module and system integration, RF transmission.
- Improving energy efficiency and energy autonomy of electronic systems through smart and intelligent battery management, energy harvesting and low leakage storage devices.
- Improved reliability, security, safety, both at software as well as hardware-level, through self-monitoring and self-repair also for harsh environments.
- Wearable and/or disposable smart systems with flexible and stretchable materials, through hybrid integration of traditional integrated circuits with flexible electronics.
- Structural electronics, opening a new form of electronics integration on/in various materials, making the package an active part of the device.
- Closer co-operation between HW, SW developers to ensure that at a system level the amount and means of processing and communications is optimized and is truly scalable and sustainable.
- Non-fossil and biodegradable materials for greener electronics and reducing environmental impact of ECS and reduction of the use of resources in general, in materials, process energy, recycling of materials.
- Quantum technologies, with components that harness quantum phenomena for computing, communication, imaging and sensing (e.g. superconducting or Si qubits, or ion traps), interfacing components and modules for quantum system integration, from cryogenic temperature (e.g. cryo-CMOS, superconducting circuitry) to room temperature.
- Miniaturization of systems at all levels to increase the performance and to save resources and materials.
- Modular systems allowing inclusion of re-used and recycled components and new high-performance building blocks for sensor-supported intelligence (e.g. sensor fusion and decision-making tasks).
- Improved system engineering efficiency using highly dynamic systems supporting automated design for easy integration of new hardware or software components / modules.
Technology advances at the component, module and system level will have a key impact on applications. Future smart components, modules and systems will show a strong increase in functional and structural complexity and higher integration levels. They will demonstrate even smaller form factors with more diverse features and materials integrated within a given volume.
Applications are the driver for such approaches:
- Communication landscape with 5G, 6G and increasing data rates, including Non-Terrestrial Networks (NTN), time-sensitive network (TSN) as well as navigation and localization, including also optical integration, components and systems for fiber networks.
- Autonomous systems– in mobility, transport, logistic, manufacturing or control of buildings and micro-grids, etc. (ensuring faster time response and decreasing the impact of human error).
- Ultra long-life time remote or difficult to access applications, e.g. structural monitoring of bridges, tunnels, civil structures requiring lower power consumption devices and supporting architectures, particularly for the autonomy of IoT edge devices, both energy autonomy and decision making at the edge.
- Healthcare landscape with applications towards prevention, assisted care, Point-of-Care devices and telemedicine, including rehabilitation, disabled assistance as well as treatment.
- The transition in mobility towards zero-emission power trains, with their significant cost and energy efficiency challenges, including energy systems for high-power charging and/or highly variable and changing conditions.
- Industry 4.0 manufacturing landscape to enable agility and autonomy, as well as energy and resource efficiency, including manufacturing up to lot-size-1.
- Overall transition from stable controlled environments to harsh environments with longer operational lifetime and variable conditions.
- Progression to scalable, fault tolerant and ultimately self-monitoring and repairable/re-configurable networks particularly for long life span applications such as structural health monitoring. Synergies with technologies such as neural networks for such adaptations should be considered.
- Sensing of environmental parameters in smart agriculture or aquaculture, in manufacturing and working places, at home and in urban areas.
- Imaging applications for security, healthcare, digital industry, (precision) agriculture, digital society (television, social media) and perception.
Electronic components, modules and systems are versatile in terms of design, size, material and composition, and thus the network of stakeholders involved in the production process of smart systems is equally complex. Europe’s supply chain for smart systems production consists of more than 6,000 large companies and SMEs33. Emphasizing and supporting ECS manufacturing can lead to increasing smart systems activity for European industry. In the ECS sector, this means about nine million jobs across Europe34. The European Chips Act is addressing these topics for a stronger European microelectronics value chain35.
The Covid-19 pandemic has revealed the vulnerability of global, distributed value chains. New models that will bring greater efficiency and more agile production processes need to be developed, and European manufacturing increased in key areas. This will ensure an effective and swift reaction to sudden market shocks as well as flexible manufacturing, accommodating shorter life cycles of products and fabrication-on-demand.
Europe is stronger in some microelectronic technologies, e.g. in MEMS sensors. MEMS technology is highly different in nature to CMOS, highly benefitting from heterogeneous integration. According to Yole Développement36, the global MEMS and sensor market (excluding RF filter modules) will almost double from US$48 billion in 2018 to US$93 billion in 2024. Assuming the same annual growth rate, the market should reach US$180 billion by 2030, with Europe supplying at least one-third to one-half of this market, with hopefully the same success in other microsystems’ markets.
Even though the European microchips manufacturing market share is only around 10 % (See =Figure F.7in Chapter 1.1.4), the European share of integrated products and ECS systems is much higher, for example in the automotive or telecom sectors. Software including firmware and middleware is an important part of the ECS systems (see Chapters 1.3 and 1.4). The system houses and OEMs also often catch the higher share of value of the final product than component providers. This emphasizes the importance of integration of ECS instead of providing or manufacturing components only.
To summarize, investing in the future of electronic components, modules and systems integration has the following strategic advantages for Europe:
- Increasing the value-added in Europe through integration components to functional systems and products, e.g. in automotive, med tech and telecom industries.
- Strengthening European economy through the generation of high-tech.
- Enabling successful Twin Transition (Green and Digital) in an economically feasible way though multifunctional smart devices.
- Ensuring European sovereignty and securing strategic Intellectual Property from European companies on advanced technologies in microelectronic ecosystem with regards to heterogeneous integration.
The following three Major Challenges are identified:
- Major Challenge 1: Enabling new functionalities in components with More-than-Moore technologies. Developing new features for sensors and actuators and smart systems via new materials and methods.
- Major Challenge 2: Integration technologies, processes and manufacturing. Identifying the system optimum regarding the interplay between components to modules and systems.
- Major Challenge 3: Sustainability and recyclability. Sustainable integration processes and recyclability of components, modules and systems to minimize their environmental impact
1.2.5.1 Major Challenge 1: Enabling new functionalities in components with More-than-Moore technologies
Physical and functional integration (PFI) considers the development of new elements and methods enabling more functionalities to be integrated physically on components, modules and system levels, in the most effective form factor. This requires interdisciplinary technology innovations as smart components, modules and systems may utilize a combination of features based on nanoelectronic, micro-electro-mechanic, thermoelectric, magnetic, photonic, micro-fluidic, optical, acoustic, radiation, radio frequency, biological, chemical and quantum principles. Further, many types of devices are to be integrated together, such as sensors, actuators, energy generators/batteries, microcontrollers/data processing devices, transceivers, as well as MEMS/NEMS, MOEMS and LAE devices. PFI requires not only the integration of physical components together, but also the co-design and integration of hardware and software, especially embedded software to create reliable and sustainable functional systems.
Physical and functional integration goes beyond the compact monolithic SoC approaches supported by the semiconductor technologies covered in the Process Technology, Equipment, Materials and Manufacturing Chapter. The challenge covers new functionalities and materials, using More-than-Moore and system in a package (SiP) technologies, where all functions are designed together to improve performance and compactness, something that also enables the heterogeneous integration of separate devices with different fabrication processes and methods. SiP is a mixed arena between the previous Process Technology, Equipment, Materials and Manufacturing Chapter and this one. In the former Chapter, SiP approaches result from the natural technological evolution of back-end semiconductor processes and are mostly related to the compact hybrid/heterogeneous integration of technologically 'homogeneous' components (e.g. chiplets) while this Chapter focuses on different integration methods of components with a higher degree of technological heterogeneity in different platforms, including their Integated Circuit carriers, such as wafer level fan-out-packages, PCB boards, printing and co-packaging of optics an electronics. This chapter concentrates on the physical integration of hardware, including computational devices into systems, while leaving the software to the Embedded Software chapter.
Given the broad range of physical scenarios they face, smart components, modules and systems need to interact with many environments:
- Well-controlled laboratory-like conditions, e.g. in vitro: lab-on-chip, organ-on-chip.
- Real-life scenarios like industrial and harsh environments (physical, chemical but also electrical, e.g. EMC) as well as in vivo: in the body (permanently in implants, temporary as in ingestibles), or on the body (wearable devices).
There is a multitude of operational issues affecting smart components, modules and systems regarding energy, performance and size. With respect to energy, for portable IoT devices, there is a need of low-power operation and provision of energy autonomy (self-powered devices or devices providing short-/medium-/long-term autonomy depending on the application) on the one hand and dealing with high-power density and thermal stress on the other. Regarding size, the optimal “minimum” size must be achieved. Heterogeneous integration and advanced packaging and interconnection technologies need to be utilized for achieving the best performance in the smallest form factor. In high end consumer electronics, high-performance and ultra-dense compact interfaces in all integration levels are needed, from chiplets and chip assembly and packaging to component and module level connectors and connections.
MEMS/NEMS development focuses on sensors and actuators that benefit from the free surfaces and volumes that MEMS/NEMS processing is able to produce in a semiconductor substrate. The former rely on new generations of inertial measurement units (accelerometers and gyroscopes) with increased performance, with or without AI support, magnetometers, pressure sensors, microphones, as well as particle sensors; the latter rely on piezoelectric, electro-statically or electromagnetic driven micro-mirrors, print heads, oscillators (membranes and cantilevers), tunable lenses, loudspeakers and piezoelectric micromachined ultrasound transducers (PMUTs). New piezoelectric materials, such as scandium aluminum nitride (ScAlN) enable new applications and improved performance for the MEMS devices, e.g. in acoustic RF filters, in optical systems such as lidars and in ultrasonic sensing.
Heterogeneous integration technologies are strongly driven by consumer applications, such as the various types of handheld devices. The manufacturer and associated supply chain for these high-volume applications are based in Asia, and so for PFI Europe needs to reinforce its supply chain of packaging solutions. The convergence between sensing and imaging domains for consumer applications, for example face recognition and AR based on consumer lidar solutions, requires co-integration of (high-speed) electronics and Integrated Photonics into compact systems. Further, wearable consumer electronics utilize flexible structures and technologies, which aim at even thinner and more flexible electronic components and systems e.g. for displays, wearables and novel human-machine interfaces (HMI). Structural and 3D electronics enable incorporating electronics in 3D surfaces and mechanical components by means of molding, additive manufacturing or laser direct structuring (LDS). Novel flexible and stretchable substrates (such as thermoplastic polyurethane (TPU) and polydimethylsiloxane (PDMS)), as well as new materials for active components, including conductive and dielectric inks, with organic materials, metal oxides, nanomaterials and 2D materials are required for realizing new applications from touch panels to RF antennas, control electronics, embedded lighting and sensors/actuators.
One of the key application drivers for the PFI of smart components, modules and systems is the IoT and its sensor nodes, which require a wide range of sensor and actuator functionalities, combined with data processing and wireless communication, and with power autonomy provided by energy storage and harvesting devices. In many cases, power autonomy is the limiting factor in such applications. This means the development of low-power solutions for sensors and actuators, as well as radio communication components and processing. Thermal management challenges introduced by increased functionality in a minimum form factor need to be solved. New and improved energy storage, especially low-leakage rechargeable storage devices, need to be developed as well as universally deployable harvesting solutions to improve the case-specific devices used today. In addition to this, it is important to improve in low-power techniques at the system level with co-design of hardware and software and overall system architecture, e.g. in wirelesss sensor networks, to ensure reliable, sustainable and energy efficient data collection and processing systems are devised. Reliable and fault-tolerant wireless networks are required for applications where long-term continued sensing is critical (e.g. structural health monitoring).
Components to provide power efficient computational resources, i.e. low-power microprocessors and devices with novel computational architectures such as neuromorphic devices) are needed, as are low-power computational methods, including distributed and low-power AI solutions in hardware, software, and in-sensor data processing. In addition, reliable, energy-efficient, low-loss interconnection and packaging solutions are a necessity.
Smart components, modules and systems leverage a multitude of materials, such as silicon and other-than-silicon semiconductors, precious and rare earth metals, ceramics, polymers, glass, inks and functional materials for sensing, actuation and energy harvesting, as well as hybrid combinations of substrates and materials (e.g. Si, ceramic, polymer, glass, metallic glass), in packages and in systems, extending the coverage of the usual materials in semiconductor-based technologies. Many of the new features required by future smart systems can only be achieved by introducing novel materials into the devices and systems, from back-end of the line processing of the microchips, or by post-processing on CMOS, to novel IC carrier technologies, including fan-out wafer level packaging and other SiP technologies as well as PCB boards or in printing, additive manufacturing or other means. The development of new materials and the compatibility of those (with regard to e.g. process compatibility, environmental compatibility) is critical to the future development of PFI.
The following key focus areas address the multimodality of ECS, which goes beyond semiconductor technology, requiring advanced packaging and heterogeneous integration of diverse components and platforms. Full coverage of the physical-functional integration requires considering both the physical integration technology platforms and the functionalities of the integrated systems.
- Sensing, imaging and actuation:
- Sensors and actuators leveraging the integration of MEMS/NEMS, MOEMS and micro-optics elements.
- Sensors and actuators for biological, medical and diagnostic applications, and for sensing of human vital signs and biomarkers, as well as for selective detection of gas and volatiles, allergens, residues in food/water, atmospheric particles, detection of substances and radiation.
- Sensors and systems utilizing quantum principles, e.g. single photon sensors, including required cryogenic and cooling components and systems.
- Devices with new features and improved performance for sensing and actuation using novel materials (metal nanowires, carbon nanotubes (CNTs), graphene and other 2D materials, cellulose nanofibers, nitrogen voids in diamond, metamaterials, metallic glass etc.), in combination or integrated with/on CMOS.
- Imaging systems: lidars and radars, including multi-modal and hyperspectral, i.e. spectrally resolved, sensors.
- Materials for affordable infrared imaging, integrated with Si (readout and image processing) electronics.
- Ultra-low power event-based sensors, e.g. inertial motion & image detection for asset tracking, incident/anomaly detection, etc.
- Materials that ensure the hermetic sealing of sensors, actuators and systems and at the same time contribute to the miniaturization of components.
- MEMS technology
- CMOS or GaN-compatible thin film piezoelectric materials, such as ScAlN for piezo-actuated MEMS sensors and actuators.
- Acoustic piezo-MEMS devices, pMUTs, and Acoustic RF filters for high frequencies above 6 GHz.
- Audio MEMS devices with more advanced integrated functionality, such as integrated noise cancelling.
- Integration of MOEMS and MEMS for novel sensors, such as hyperspectral imagers.
- Integrated Photonics
- New materials for active photonics devices, such as 2D materials, Indium Phosphide; for improved performance, such as higher bandwidth in modulators, and detectors.
- Laser sources, with higher power and better performance and with tunable wavelength using external cavity on photonic integrated circuits (PIC).
- New waveguide materials and components for new wavelengths, e.g. at UV and mid-IR.
- New devices for Quantum PICs.
- Flexible electronics
- Sensing devices and power sources compliant with hybrid integration in wearables, considering flexibility, durability (e.g. washability) and biocompatibility.
- Flexible and stretchable sensors and modules, e.g. OLED displays, OPVs, touch surfaces and other sensors/actuators, conformal antennas.
- Functional materials for flexible and stretchable devices; organic and inorganic semiconductor materials and inks, perovskites for OPV.
- Barrier materials, dielectrics, and transparent conductor materials and inks for flexible electronics and additive manufacturing.
- Communications:
- Module-level high-speed wireless communication features, including current and new frequency bands.
- High-speed photonics communications modules beyond 1 Tb/s.
- New front-end components, filters and functionalities e.g. active antennas for 5G and 6G communications and non-terrestrial network solutions.
- Low latency and low power communications in-package/module as well as at system level for the edge and IoT devices.
- Continuous delivery of new features and fixes through Over-The-Air (OTA) updates to ensure the security of the device over time and reduce the digital waste increasing the life of a device.
- Strategies and components for Electromagnetic interference (EMI) mitigation and reliable operation in harsh environmental conditions.
- Energy and thermal management:
- Low-power/low-loss modules for low-power sensing, actuation, processing and communication.
- Energy-autonomous multi-sensor modules and systems including sensing, actuation, processing and communication.
- Power management components and modules compatible with harsh environments (high temperatures, vibrations, electromagnetic interference (EMI) conditions for industrial, automotive and space technology).
- Devices using non-toxic materials for efficient energy sources, storage and harvesting devices (thermoelectric, piezoelectric, tribo-electricity, etc.), and higher performing electrodes and electrolytes for improved capacity and low leakage for energy storage devices.
- Solutions for thermal management for integrated photonics and RF systems and at different integration levels including advanced and active cooling systems.
- Thermal management and smart cooling systems for industrial applications and harsh environments.
- Efficient smart compact cooling solutions and approaches for quantum devices and cryogenic multiplexing with semiconductors or superconducting devices.
- Simulation models at device and system level to guide system level efficiency optimization and to help make informed decisions for component selection and data procession and comms architectures.
- Information processing:
- Component and system-level features for self-diagnosis and module-level signal processing and control features for self-diagnosis, self-monitoring, and self-learning and self-repair.
- Sensor level hardware and software solutions for security and privacy and data trustability.
- Machine learning and artificial intelligence and data analysis on the sensor, module and systems level, i.e. on the edge data analysis embedded on different levels for smarter devices, including AI on the sensor level.
- Integrated and scalable solutions, both Software and hardware integration, especially for edge AI.
- Use of quantum computing and integrating quantum computing for data-analysis.
1.2.5.2 Major Challenge 2: Integration technologies, processes and manufacturing
Smart components, modules and systems require a multitude of processes: silicon and other micro- and nano-processing, additive manufacturing, lamination and other interconnection and assembly technologies, as well as hybrid combinations. To increase the integration density and combine the above-mentioned features, many different integration and packaging technologies are required, such as thin film processes, embedding, classic assembly and joining methods and additive manufacturing, both for single components as well as modules.
Heterogeneous integration, from components up to the systems level, requires-engineering on many technology domains, such as power, signal integrity, EMC, thermal, mechanical. All such domains must be designed together to ensure a high level of performance and the necessary integration. The challenge here is to combine all these domains in the design and simulation of integrated systems, often with inadequate information of all the properties of the included materials, components and processes. Furthermore, design and simulation methods that enable and support such multi-physics and multimodal design and manufacturing must be addressed, with possibility to parameterize not only the material parameters, but also system parameters, e.g. variability in the quality of the contact (e.g. thermal, mechanical) between the transducer and the ambient energy sources in the case of power harvesting. Modelling and design tools for thermal, mechanical and electrical characteristics in small 3D packages, including molded and additive manufacturing methods are needed, linking to Architecture and Design; Methods and Tools Chapter.
Flexible electronics is an enabler to reduce the weight, volume and complexity of integrated systems and products, to create novel form factors and 3D design features. Currently, the majority of flexible electronics products are based on polyimide (PI, Kapton), copper laminate substrates, etching of copper to pattern the circuitry, and conventional SnAgCu (SAC) soldering or anisotropic conductive adhesives (ACA) bonding processes for the assembly of discrete components on the substrate. Development towards smaller feature size in printing technologies increases the requirement of registration, or layer-to-layer alignment accuracy. Development of IC interconnection and bonding technologies especially to flexible and stretchable substrates is critical for improved performance, yield and reliability. In addition, pilot lines and fabrication facilities and capacities need to be developed.
In integrated photonics, the optical waveguides and devices are fabricated as an integrated structure onto the surface of a substrate, typically Silicon wafer, but also other substrates. As a result of integration, complex photonic integrated circuits (PICs) can process and transmit light in similar ways to how electronic integrated circuits process and transmit electronic signals. New waveguide and active materials are constantly developed, both for monolithic integration of components, such as SiN waveguides on Silicon or Ge detectors and 2D materials for active components, such as modulators or detectors) and heterogeneous integration of active components on the PICs. The fabrication of PICs consists of a multi-faceted integration problem, including monolithic integration, heterogeneous integration of active components (e.g. laser sources), and high-speed driving electronics for e.g. high-speed communications above 100 GHz bandwidth, thermal management and other functionalities, such as fluidic functions for bio and medical sensing.
The critical requirements for any of these techniques to enable new advanced applications are to ensure sustainable and cost-efficient manufacturing while providing high performance and reliability. Further important developments include integration of different silicon IC components into miniaturized multifunctional modules following different SiP approaches, combining technologies such as flip chip, bonding, lamination and substrate materials such as silicon, glass, ceramics and polymers. Multifunctional integration also requires the development of multi-domain integration – e.g. the integration of photonic and RF functionalities into smaller form factors and together with sensors and CPUs.
The challenge of integration processes, technologies and the manufacturing of smart components, modules and systems is mainly about dealing with the complexity of heterogeneous integration and scalable manufacturing technologies with different economy of scale approaches. These include “intensive” Si-like technologies, or “extensive” printing-like technologies, which under different assumptions and processing paradigms can offer cost affordability and production scalability. Apart from high-volume applications such as medical patches and RF front-end modules for 5G/6G small cells, some industrial applications can also require the availability of components, modules and systems in relatively small quantities over decades, which adds a new challenge to the scalability of manufacturing and implementation of the latest technologies.
The complexity and diversity of heterogeneous components, modules and systems substantially exceed that of mere microelectronic components due to their multi-physics and multiple domain nature. In addition, the packages will include integrated functionalities, rather than being “passive” boards and frames. Integration and packaging methods should increase, not decrease, the performance of the interlaying component. Especially low-loss integration methods to enable integration of large RF systems or integrated photonics. These technologies would enable e.g. RF front ends or active antennas for millimeter wave frequencies enabling novel beyond-5G telecom solutions, or in integrated photonic communication and sensing systems. Further co-integration and co-packaging of high-speed electronics and integrated photonics is needed.
In this multifunctional and multimodal integration on the component, module and system level, the development of manufacturing methods that meet the accuracy and repeatability criteria of high-quality and high-reliability products for a broad range of applications and constraints (physical, mechanical, thermal, environmental) is challenging and needs development.
Additive manufacturing can provide structural and functional solutions for smart components, modules and systems integration that are not feasible with traditional methods. These methods will enable zero-defect manufacturing starting at lot one. Although additive manufacturing also improves manufacturing flexibility, solutions for the cost-efficient scaling of these fabrication methods must be addressed. 3D component, module and system integration methods will need to be developed to provide greater functionality and miniaturization in a cost-effective and scalable way.
With respect to this multi-modality of heterogeneous integration methods, the key focus areas are divided based on the main technologies: advanced packaging and SiP technologies, integrated photonics, flexible electronics, quantum systems and manufacturing methodology as follows.
- Advanced packaging and SiP technologies
- Robust heterogeneous 3D integration of sensors, actuators, electronics, communication, RF front-end components and energy supply into systems.
- Embedding of power sources (energy harvesting transducers, batteries, supercaps, etc.) into a package (PwrSiP) and on a chip (PwrSoC).
- IC carriers with integrated voltage regulator and capacitance increasing the power delivery efficiency, as well as finer structuring in IC carriers: below 5/5µm line width and spacing and finer micro-vias (below 15µm diam.).
- Integration with biological and molecular systems, including fluidics and surface coatings and functionalization materials and methods for multi-functionality on the same base structures, e.g. biosensor arrays on Silicon.
- New functional materials for packaging that enable integration of sensing or other functionality into the packaging itself, e.g. packaging as a part of the antenna or sensor functionality.
- New materials and methods for housings and coating features and new substrate materials for specific requirements: high power, high frequencies, disposable, bio-compatible, non-fossil, harsh environments.
- Rapid prototyping and manufacturing technologies (additive manufacturing, 2D and 3D additive technologies, etc.).
- High-performance materials for passives enabling close coupled passives for high density heterogeneous integration such as magnetic cores, high-k dielectrics.
- Ultra-dense and small interfaces in all integration levels, from chip assembly and packaging to component and module level connectors and connections.
- Integration of different sensors and sensor hubs for sensor fusion (e.g. combination of acceleration sensor, microphone, microspeaker for enhanced noise cancellation).
- Manufacturing and characterization processes for hermetic sealing of com-ponents or subsystems with low leakage level.
- Integrated Photonics and co-integration with electronics
- Photonic-electronic system integration based on integrated photonics, including high-speed RF electronics, MEMS/NEMS sensors, etc.
- Multi-domain electro-photonic integration and electro-optic co-packaging.
- Enabling electronic-photonic systems by heterogeneous integration of active components on PICs (III-V semiconductors, ferroelectrics, ultra-low-loss waveguide materials).
- Heterogeneous integration processes and equipment for integrated photonics, including high-precision component placement and bonding, as well as low-loss fiber coupling to PICs.
- Flexible electronics
- Integration towards low vertical form factor (<100 μm) and the miniaturization of external matching networks through integration.
- Submicron LAE fabrication processes and equipment (printing technologies in general, nanoimprinting, reverse offset printing, etc.) and automated manufacturing equipment for flexible electronics, including testing tools for electrical and non-electrical properties.
- Interconnections processes and tools for flexible and stretchable devices and structural electronics (in glass, plastics, laminates, etc.).
- New/alternative non-fossil, organic, biocompatible and compostable substrate materials for e.g. implants, ingestibles, wearables, biosensors.
- Adhesives, bonding materials and methods for integrating chips on flexible substrates.
- Use of flexible Si-substrates for 3D form factors and for flexible electronics.
- Quantum systems
- Materials and methods for integration and packaging of semiconductor electronics, Integrated Photonics and superconducting devices at cryogenic temperatures, including 3D technologies.
- Integration and interfacing and cabling solutions for combining room temperature systems and cryogenic quantum components, sensors and systems.
- Integration methods that enable scaling quantum systems efficiently, from wafer level 3D integration to module and system level.
- Manufacturing methodology, characterization and testing
- Automation and customization in component, module and system integration for large-scale manufacturing, including Industry 4.0 techniques, design for manufacturing based on production data techniques, and lot-size-1 manufacturing.
- Manufacturing and testing tools (including tests, inspection) for components, modules and systems, enabling zero-defect integration.
- Material properties database for simulation and reliability.
- Design of new materials from properties requirements by the means of Materials by design, materials genome and digital design approach.
- New selective heating processes (i.e. inductive or reactive) to limit overall temperature impact to system while packaging.
1.2.5.3 Major Challenge 3: Sustainability and recyclability
As increased integration will cause the borders between components, modules and systems to become blurred, and more diverse and complex materials are used at each level, the dismantling of systems into their constituent components at the end of their useful life will become increasingly difficult. Many industrial ECS products have life times extending to decades, thus the environmental regulations for recyclability cannot be known at the time of product design in detail. Incineration is traditionally the only “recovery” strategy used to dispose of an integrated system once it is discarded.
ECS should produce smart systems not only as an enabler for, but also as an element of the circular economy, considering the sustainability of the ECS value chain and the products themselves. Focus should be on the sustainability of the component, module and system production, including processes, materials and maintenance during the primary life time. The recyclability of the product must be considered already in the design and manufacturing phase to enable repairability, upgradeability, extension of life time, and re-use in a second life application, and finally the recovery of components and materials for recycling.
In 2019, world-wide e-waste exceeded 50 million tons, and it is forecasted to grow to 70 million tons in 203037. Given the increasing burden of improperly dealt with e-waste and considering that a significant part of CO2 emissions arises from the fabrication of the ECS themselves, extending product life time is important for reducing ECS-related environmental load. This needs to be addressed by designs that enable repair or replacement of faulty components instead of replacing the full module or system. To fight obsolescence, hardware and software upgrades should be supported, even in field conditions. Reducing CO2 emissions during the life time of the system requires minimizing the power consumption at component, module and system levels while in operation by using low-power hardware and software technologies.
Future ECS products must be environmentally friendly, covering all aspects from materials, manufacturing, operation and maintenance during their life time, considering recycling at their end-of-life. Activities must start by ensuring recyclability (eco-design, environmentally friendly materials and manufacturing), employing a low CO2 footprint over the whole lifecycle, facilitating the transition to a circular economy, wherever possible. Activities will be on:
- Upstream considerations and design for repair, upgradeability, dismantling, materials separation and recycling.
- Non-fossil, recyclable, biodegradable and compostable materials, without releasing any dangerous materials or having other negative impact on environment.
- Life time extension and system health monitoring, self-monitoring and healing.
- Increasing energy efficiency of ECS, during manufacturing and life time, and end-of-life.
- (Predictive) maintenance, repair, upgrading, reconfiguring, recharging, retrofitting and re-use in second life, including ecosystems and tools to support these actions.
- Sustainability and reducing energy consumption and environmental footprint of the manufacturing and integration processes.
The development of integration processes based on new design tools will allow the dismantling of components, their recycling and the recovery of materials (urban mining). Therefore, system design techniques need rethinking to use multifunctional components and modules, and design for component separation and recyclability is generally required, in selection of materials and in integration technologies. Recycling technologies, as well as new approaches to second life of ECS and re-use in new application, must be advanced. For example, with the electrification of cars, the recycling and re-use of battery packs, modules, and individual cells, and finally, materials recovery from the cells, becomes more and more important.
The use of new environmentally friendly, recyclable and non-fossil materials (or compostable/biodegradable materials) must be seriously considered to replace existing materials with low recyclability in the near future. The use of these materials can easily be extended to other parts of the system, and the development of biodegradable materials can also contribute to solving the problems of recyclability. Life cycle assessment (LCA) should be used as a design tool to minimize the ECS carbon and environmental footprint, considering the full life cycle and end-of-life. Finally, we should of course minimize the number of batteries we use and dispose and improve recycling of batteries and battery materials.
- Sustainable integration, manufacturing and use of ECS:
- Modular design for repairability and maintenance and updating. Condition monitoring for usage as well as for health.
- Optimization of resources and processes in production environment with potential in-situ re-use and regeneration of base materials and chemicals, and reducing energy consumption to lower greenhouse gas emissions.
- Increasing power efficiency of the ECS during life time by using low-power techniques.
- Condition monitoring and predictive maintenance as tools to extend life time of ECS, including associated pertinent physics of failure models.
- Assessment of environmental impact ECS in design stage, as a tool for sustainable ECS, using life cycle assessment (LCA) or similar framework.
- Recyclability of components, modules and systems:
- Design tools for optimized use for materials.
- Use of recyclable, biodegradable, compostable, non-fossil materials.
- Designing and developing integration processes that allow module dismantling, component recycling and material recovery (urban mining).
- Recycling technologies (e.g. for precious metals), second-life scenarios, repair, re-purposing and re-use approaches.
- Breakthroughs and development in recycling process and solutions for energy storage components, such as batteries.
- Replacement materials to comply with Restriction of Hazardous Substances Directive (ROHS) regulations and minimize critical raw materials (CRM) dependence, including rare earths replacement for magnetics, inductors and power integrity.
The following table illustrates the roadmaps for Components, Modules and Systems Integration.
MAJOR CHALLENGE |
TOPIC |
SHORT TERM (2023-2027) |
MID-TERM (2028-2032) |
LONG TERM (2033 AND BEYOND) |
Major Challenge 1: |
Topic 1.1: Sensing, imaging and actuation |
|
|
|
Topic 1.2: MEMS technology |
|
|
|
|
Topic 1.3: Integrated photonics |
|
|
|
|
Topic 1.4: Flexible electronics |
|
|
|
|
Topic 1.5: Communications |
|
|
|
|
Topic 1.6: Energy and thermal management |
|
|
|
|
Topic 1.7: Information processing |
|
|
|
|
Major Challenge 2: Integration technologies, processes and manufacturing |
Topic 2.1: Advanced packaging and SiP technologies |
|
|
|
Topic 2.2: Integrated Photonics and co-integration with electronics |
|
|
||
Topic 2.3: Flexible electronics |
|
|
|
|
Topic 2.4: Quantum systems |
|
|
|
|
Topic 2.5: Manufacturing methodology, characterization and testing |
|
|
|
|
Major Challenge 3: Sustainability and recyclability |
Topic 3.1: Sustainable integration and use of ECS |
|
|
|
Topic 3.2: Recyclability of components, modules and systems |
|
|
|
Smart components, modules and systems are key elements in a wide range of activities relevant to all Application Chapters. Conversely, the new and advanced applications described in those chapters will also give rise to new functionalities and further advances in integration technologies. Most components, modules and systems integration is based on devices developed with techniques described in Process Technology, Equipment, Materials and Manufacturing Chapter. Furthermore, simultaneous development and co-design is necessary with Embedded Software and Beyond technologies to ensure integration with hardware and software.
The cross-sectional technologies link to components, modules and system integration in many ways. Connectivity solutions are needed for networked systems. Edge Computing and Embedded Artificial Intelligence needs to link into integrated systems for AI on the edge and on sensor level already. Quality, reliability and cybersecurity methods are paramount for ensuring reliable integrated systems. For successful multi-modal integration of electrical, thermal, and mechanical properties in integrated systems, advanced simulation methods and design tools are required, which is covered in Architecture and Design: Methods and Tools.
Thus, the field of components, modules and systems integration draws upon key enabling technologies and integrates knowledge from many disciplines. In addition, integration bridges the gap between components, modules and functional, complex systems. As the development of smart components, modules and systems will benefit from progress in all other technological disciplines, the synergies should not only be in the multidisciplinary development of the technologies, but also in the building of ecosystems (people and infrastructure). This is where all stakeholders can guide and influence each other and collaborate to assist in the development of optimized system- and application-oriented solutions.
33 Prognos AG: Analyse zur ökonomischen Bedeutung der Mikrosystemtechnik, Studies about the Smart Systems economy in Baden-Württemberg and Germany; European Competitiveness Report; EU Industrial Structure 2011; Figures provided by major industry associations
34 EU Commissioner Gabriel at the ECSEL JU Symposium 2020 (https://www.ecsel.eu/ media/2081)
35 https://digital-strategy.ec.europa.eu/en/library/european-chips-act-communication-regulation-joint-undertaking-and-recommendation
36 Yole Développement: Status of the MEMS Industry 2019, Market and Technology Report, 2019 edition
37 Forti V., Baldé C.P., Kuehr R., Bel G. The Global E-waste Monitor 2020: Quantities, flows and the circular economy potential. United Nations University (UNU)/United Nations Institute for Training and Research (UNITAR) – co-hosted SCYCLE Programme, International Telecommunication Union (ITU) & International Solid Waste Association (ISWA), Bonn/Geneva/Rotterdam.